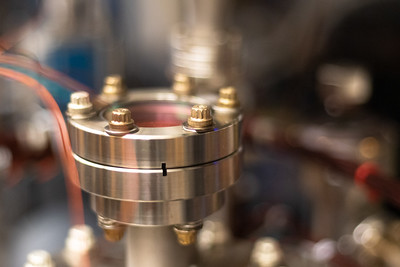
When light travels through a cloud of atoms, some photons are absorbed (exciting atoms, which later re-emit this energy as fluorescence, in all directions) while others are transmitted. With modern techniques, it is possible to probe the effect of a single photon on the atoms as it propagates through. While it can be shown that the total time atoms spend in the excited state is equal to the number of absorbed photons times the atomic lifetime, there has not yet been any prediction of how this time is split between cases where the photon is transmitted and those where it is “scattered” by the medium. In my PhD, I carried out the first experiment to address this basic question about how light and matter interact, and found that even photons which are not absorbed by the atoms cause the latter to spend some time in the excited state; implying in turn that absorbed photons cause atoms to spend less than their natural lifetime in the excited state before re-emitting.
When light passes through an ensemble of atoms, it can excite them, which at the macro-level results in a change in the optical properties of the ensemble. In this way, laser beams overlapped inside such an ensemble can be engineered to effectively "interact". A particularly useful interaction involves one laser beam acquiring a phase shift from the atoms which depends linearly on the number of photons in the other beam. If this phase shift can be accurately measured, it reveals the number of photons in the other beam without having to absorb them as is usually required in conventional photon counting. Unfortunately, the effect of a single photon on an ensemble of atoms in most circumstances is simply too small to be resolved. In this work, we used Rydberg super-atoms to try and enhance the effect a single photon can have on an ensemble. When a single photon excites a Rydberg super-atom, all the atoms making up the super-atom are affected, amplifying the phase shift per photon. This was the first result from the new Rydberg apparatus, which I built in the Steinberg lab during my PhD and marks the start of a new research program for the group.
Quantum theory does not have a clear ontology that associates observables with an objective, underlying reality. Formally, we cannot make definite statements about observable quantities such as the number of photons passing through an arm of an interferometer or similarly the intensity of the electric field in that arm. Instead, we can only make predictions about the possible results of measurements that inherently disturb the system (or at least its quantum state). Ontological theories are difficult to construct in the general case, so a good starting point for considering possible ontologies are models that apply in particular cases, but may not (or explicitly do not) apply in others. Here, we apply such a model to a recent experiment and show that the experimentally measured values correspond to the average of the ontological entities (the electromagnetic field intensities) in the model.
Weak-value amplification (WVA) is a technique that has been used in a variety of experimental settings to permit the precise measurement of small parameters. Whether or not WVA has an actual advantage in terms of the resulting measurement precision has been the subject of an ongoing debate over the past few years. In this paper, we delineate the situations under which WVA does in fact improve measurement precision in the presence of correlated noise, comparing it with competing approaches. This investigation leads to interesting connections between WVA and other techniques such as background subtraction and lock-in amplification, which elucidates the technical advantages that have already been experimentally observed.
In quantum mechanics, the concept of weak measurements allows for the description of a quantum system both in terms of the initial preparation and the final state (post-selection). This paradigm has been extensively studied theoretically and experimentally, but almost all of weak-measurement experiments carried out to date can be understood in terms of the classical (electromagnetic wave) theory of optics. Here, we present a quantum version in which the measurement apparatus deterministically entangles two distinct optical beams. We show that a single photon, when properly post-selected, can have an effect equal to that of eight photons: that is, in a system where a single photon has been calibrated to write a nonlinear phase shift of X on a probe beam, we measure phase shifts as large as 8X for appropriately post-selected single photons.
Photos of quantum optics and atomic physics labs at MIT and Harvard taken by @sinclairjosiah.